Sulfur And The Origin Of Life
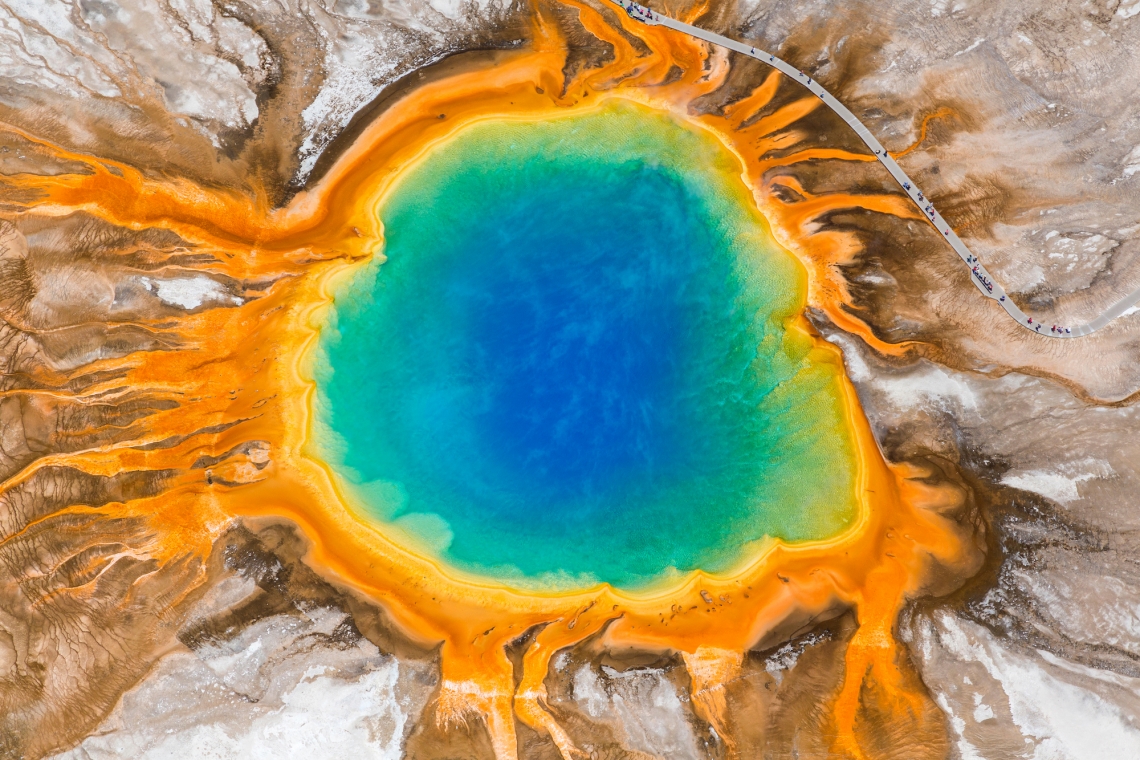
Many artists have tried to depict what Earth might have looked like billions of years ago, before life made its appearance. Many scenes trade snow-covered mountains for lava-gushing volcanoes and blue skies for lightning bolts pummeling what’s below from a hazy sky.
But what did early Earth actually look like? This question has been the subject of intense scientific research for decades.
A publication led by Sukrit Ranjan, an assistant professor in the University of Arizona’s Lunar and Planetary Laboratory, shines a spotlight on sulfur, a chemical element that, while all familiar, has proved surprisingly resistant to scientific efforts in probing its role in the origin of life.
“Our picture of early Earth is pretty fuzzy,” said Ranjan, who explores sulfur concentrations in early Earth’s waters and atmosphere. The same processes that make our planet habitable – liquid water and plate tectonics – constantly destroy the rocks that hold Earth’s geologic record, he argues. “It’s great for us because it recycles nutrients that would otherwise be locked up in Earth’s crust, but it’s terrible for geologists in the sense that it removes the messengers.”
Published in the journal AGU Advances in December, Ranjan’s paper was selected as an editor’s highlight, in recognition of “experiments that were extremely difficult to perform but provide constraints for ongoing laboratory prebiotic chemistry experiments.”
At the core of efforts to pull back the curtain on the emergence of life on Earth has been a concept known as the “RNA world,” Ranjan said, referring to ribonucleic acid, a class of molecules that are present in every living cell and crucial to life as we know it.
The RNA world hypothesis is based on an interesting feature of modern biology, which is that of the four major categories of biomolecules – amino acids, carbohydrates, lipids and nucleic acids – RNA is the only one that can perform the role of an enzyme and the storage and replication of genetic information, by making copies of itself, all by itself. There’s just one problem: It’s really hard to make.
“For about 50 years, people have tried to figure out how to make RNA without enzymes, which is how biology does it,” Ranjan said, explaining that it wasn’t until the last five years that researchers figured out non-enzymatic pathways to make RNA.
“If we can get RNA, then on the far horizon we see a pathway to get everything else going,” he said. “And this begs the question: Was this molecule actually available earlier in any quantities whatsoever? And this is actually a major open question.”
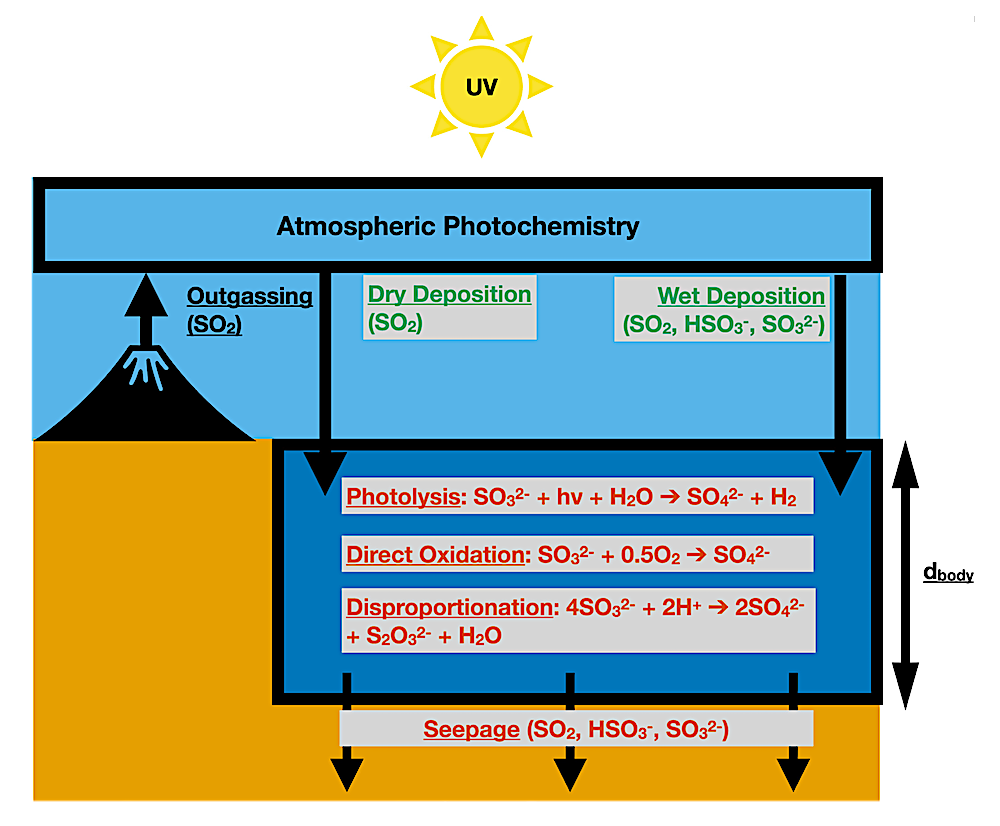
Schematic illustrating our model of S[IV] in natural waters on early Earth. Input of S[IV] from the atmosphere (ultimately derived from volcanic outgassing) into an aqueous body (dark blue box) via wet and dry deposition is balanced by loss of S[IV] in the aqueous body due to photolysis, direct oxidation, disproportionation, and seepage. Processes that are sources of aqueous S[IV] in the 0D aqueous photochemistry box model are in blue-green text, while processes that are sinks of aqueous S[IV] in the 0D aqueous photochemistry model are in vermillion text. Specification of geochemical parameters allows simulation of both marine and terrestrial waters using this modeling approach.
Recently, scientists have completed a half-century quest to make RNA molecules without biological enzymes, a huge step forward to demonstrating the RNA world. However, these chemical pathways all rely on a critical sulfur molecule, called sulfite. By studying rock samples from some of Earth’s oldest rocks, scientists know there was plenty of sulfur to go around on the early, prebiotic Earth. But how much of it was in the atmosphere? How much of it ended up in water? And how much of it ended up as RNA-producing sulfite? Those are the questions Ranjan and his team set out to answer.
“Once it’s in the water, what happens to it? Does it stick around for a long time, or does it go away quickly?” he said. “For modern Earth we know the answer – sulfite loves to oxidize, or react with oxygen, so it’ll go away super-fast.”
By contrast, as geological evidence indicates, there was very little oxygen in early Earth’s atmosphere, which could have allowed sulfite to accumulate and last much longer. However, even in the absence of oxygen, sulfite is very reactive, and many reactions could have scrubbed it from the early Earth environment.
One such reaction is known as disproportionation, a process by which several sulfites react with each other, turning them into sulfate, and elemental sulfur, which are not useful for origin-of-life chemistry. But how fast is this process? Would it have allowed for sufficient quantities of sulfites to build up to kickstart life?
“No one has actually looked into this in depth outside of other contexts, mainly wastewater management,” Ranjan said.
His team then set out to investigate this problem under various conditions, an effort that took five years from designing the experiments to publishing the results.
“Of all the atoms that stock the prebiotic shipyard, including carbon, hydrogen, nitrogen, oxygen, phosphorus and sulfur, sulfur is perhaps the thorniest,” wrote Sonny Harman of NASA’s Ames Research Center, in a viewpoint article accompanying the publication. Because of its eagerness to enter into chemical reactions, “sulfur compounds tend to be more unstable, posing hazards to lab personnel and equipment, clogging up instrumentation and gumming up experiments.”
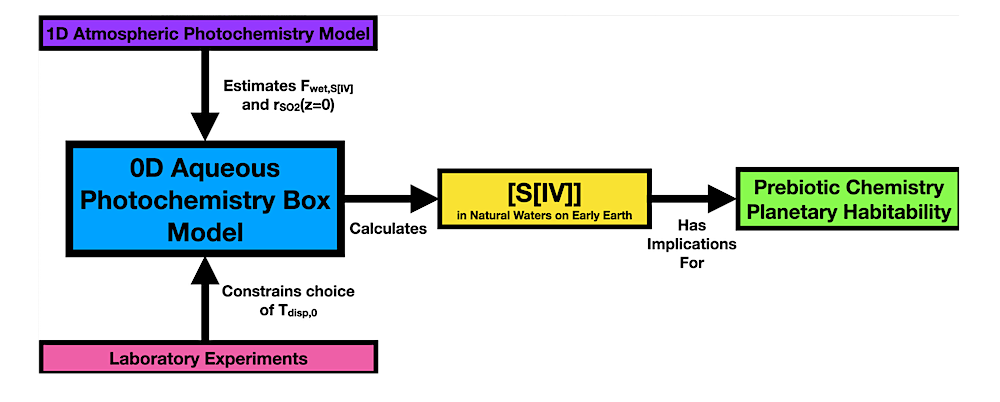
Schematic showing how the modeling and experimental work presented in this paper fit together. The core of the work is the novel 0D S[IV] aqueous photochemistry box model, from which our scientific conclusions are obtained. The purpose of our laboratory experiments and atmospheric photochemical modeling is to set input parameters for the aqueous photochemistry box model. The box model is then used to estimate [S[IV]] in marine and terrestrial natural waters on prebiotic Earth, and then implications for prebiotic chemistry and planetary habitability are evaluated.
A lab tech’s nightmare
In their setup, Ranjan and his co-authors dissolved sulfite in water at various levels of acidity or alkalinity, locked it into a container under an oxygen-free atmosphere and let it “age,” as Ranjan put it. Every week, the team measured the concentrations of various sulfites with ultraviolet light. At the end of the experiment, they subjected them to a suite of analyses, all geared toward answering a relatively simple question, he said: “Just how much of this original molecule is left, and what did it turn into?”
Sulfites, it turned out, disproportionate much slower than what conventional wisdom held. Earlier studies, for example, had floated the idea of a sulfur haze engulfing the early Earth, but Ranjan’s team found that sulfites break down under ultraviolet light more quickly than expected. In the absence of an ozone layer during Earth’s early days, this process, known as photolysis, would have quickly purged sulfur compounds from the atmosphere and the water, albeit not quite as efficiently as the abundant oxygen in today’s world.
While it’s plausible that slow disproportionation could have allowed sulfites to accumulate, photolysis would have made that very unlikely except in certain environments such as shallow water pools, shaded from UV radiation, particularly if fed by surface runoff to provide mineral shields. Examples include underground pools or closed basin carbonate lakes, drainage-less depressions where sediments accumulate but water can only leave by evaporation.
“Think bodies of water like the Great Salt Lake in Utah or Mono Lake in California,” Ranjan said, adding that hydrothermal environments are emerging as hot candidates for life’s first appearance. Here, groundwater carrying dissolved minerals comes into contact with heat from volcanic activity, creating unique micro-environments that offer “safe spaces” for chemical process that could not occur elsewhere.
Such places can be found at mid-ocean ridges in the deep sea, but also on land, Ranjan said.
“A modern-day example of this is Yellowstone National Park, where we find pools that accumulate lots of sulfite, despite the oxygen,” he said, “and that can happen just because the sulfite is continually being replenished by volcanic outgassing.”
The study provides opportunities to test the hypothesis of sulfite availability in the evolution of the first molecules of life experimentally, the authors point out. Ranjan said one field of research in particular has him excited – phylogenetic microbiology, which uses genome analysis to reconstruct the blueprints of sulfur-using microorganisms believed to represent the oldest phyla on Earth.
There is evidence that these bacteria gain energy by reducing highly oxidized forms of sulfur to less oxidized ones. Intriguingly, Ranjan pointed out, they depend on a fairly complex enzyme machinery for the first step, reducing sulfate, sulfur’s abundant “modern” form, to sulfite, suggesting these enzymes are the product of a long evolutionary process. In contrast, only one enzyme is involved in the conversion from sulfite – the proposed key ingredient in “prebiotic puddle environments” – to sulfide.
“If true, this implies that sulfite was present in the natural environment in at least some water bodies, similar to what we argue here,” he said. “Geologists are just now turning to this. Can we use ancient rocks to test if they’re rich in sulfite? We don’t know the answer yet. This is still cutting-edge science.”
Geochemical and Photochemical Constraints on S[IV] Concentrations in Natural Waters on Prebiotic Earth, AGU Advances (open access)
Astrobiology,